A Self-Propelled Compass Needle: Places to Be, Bacteria to See!
- Steph Calridge
- Sep 7, 2022
- 6 min read
Updated: Sep 14, 2022
Magnetotaxis and magnetoreception are abilities present across both the Earth and its phyla due to the planet’s ever-present magnetic field. These species – such as bacteria, microbial eukaryotes, birds, molluscs, and reptiles — use the magnetic field to navigate during large migrations or for small movements, thus allowing species to inhabit more favourable environmental conditions. In eukaryotic microbes, this ability has been assumed to result from symbiotic relationships with magnetotactic bacteria (MTB), while the origin of magnetotactic abilities in macroeukaryotes has remained largely unknown. One study [1] suggests that similar symbioses are a common occurrence among magnetotactic macro-eukaryotes. However, the recent discovery of microbial eukaryotes that can biosynthesise components necessary for magnetotaxis (rather than uptaking them from the environment or engulfing magnetotactic bacteria to form symbiotic relationships) will challenge current understandings, and add to a wide variety of bacterial biomedical and nanotechnological opportunities.
The Ocean is Full of Tiny Microbial Compass Needles
A range of microbes use Earth’s magnetic field in orienting and navigating themselves toward anoxic environments; this is often paired with aerotaxis, a movement and directionality driven by oxygen availability. Many microbes move through random Brownian movement1 , but this magnetotaxis directs flagellated microbes towards anaerobic and micro-aerobic sediments2 . This ability arises from the magnetosome. This organelle is present in two forms, each with a lipid bilayer membrane: an iron oxide magnetosome with a magnetite crystal or an iron sulphide magnetosome with a greigite crystal [2]. In prokaryotes, these magnetosomes are bulletshaped or prismatic and form chains in the cytoplasm along a dipole (thus creating a “compass needle”) (Fig. 1).
Magnetotaxis is not confined to one bacterial clade but rather has developed across many taxa. Because of this, MTBs are globally distributed and have been found across a wide range of environments (many of which qualify them as extremophiles). The presence of MTBs in extreme environments — for example, as alkaliphiles [4] and thermophiles [5] — suggests that magnetosomes can form and tolerate a large range of environmental extremes.
Typically, MTBs are anaerobic or microaerobic and occupy both marine and freshwater environments and sediments. Rather than being dependent upon iron-rich environments (for the formation of the magnetite and greigite) MTBs tend to require an oxic-anoxic interface (OAI), such as those that form at the sediment-water interface. The highest concentrations have been found in the OAI — typically the top 1-4cm of sediment — although some MTBs have been identified at the water-sediment interface in oxic environments [6]. Magnetite-producing MTBs are concentrated around the OAI, while greigite-producing MTBs occupy habitats below the OAI in the sulfidic anoxic zone. This segregation is due to high concentrations of sulphide in the anoxic zone, promoting the formation of greigite (Fe3 S4 ), while the OAI has greater inputs of oxygen and thus forming magnetite (Fe3 O4 ) [7].
The reliance upon and interaction with the Earth’s magnetic field by certain bacteria creates three classifications of MTB. North-seeking MTB dominate the northern hemisphere, while south-seeking MTB dominate the southern hemisphere (and the equator has a roughly equal population of each). When inhabiting chemically and vertically stratified waters, anaerobic MTBs will swim away from the magnetic field and thus downwards to anoxic sediments. However, there are exceptions to this, as populations of north-seeking MTB are present in the southern hemisphere and vice versa. The reason for this discrepancy was initially credited as an increasing redox potential [8], but further study is required to understand the relationship between MTB densities and other abiotic factors [9].
The Independent Microbial Eukaryotes That Don’t Need No MTB
Until recently, magnetosomes have only been found in bacteria, and any magnetotactic abilities in eukaryotes were thought to be the product of ectosymbiotic relationships with bacteria and magnetosomes. These relationships occur by non-flagellated bacteria³ latching onto the surface of the eukaryote, and thus granting magnetotactic abilities to the eukaryote [10]. However, a deep-ocean foraminifera — Resigella bilocularis — has been identified and distinguished as one of the few known eukaryotes capable of biosynthesising magnetosomes without a symbiotic relationship with an MTB [11]. This protist inhabits the depths of the Mariana Trench and produces magnetite that is morphologically distinct from that produced by MTB or found in nearby sediments. The magnetite of R. bilocularis is porous, octahedral, and of varying sizes (11, Fig. 2), while the magnetite of MTB is typically smooth-surfaced, cuboidal, and arranged in one or two chains [12]. Environmental magnetite also differs because it typically has an irregular (but smooth) shape of a larger size and lacks an organic envelope. Another study [13] found a similar magnetotactic protist that likely biomineralised bullet-shaped chains of magnetite magnetosomes within its cell. However, while this evidence supports the biomineralisation of magnetite in eukaryotes, it is possible that an endosymbiotic event with MTB occurred early in their evolutionary history. Further research is required into the evolutionary history and relationships of these protozoa and bacteria.
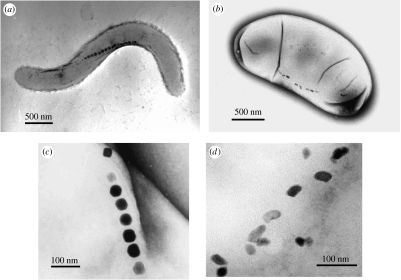
Figure 1: This shows transmission electron microscope images of different magnetotactic bacteria. The magnetite magnetosomes are the black lines, often seen in a single line. Scale bars represent 100 nm.
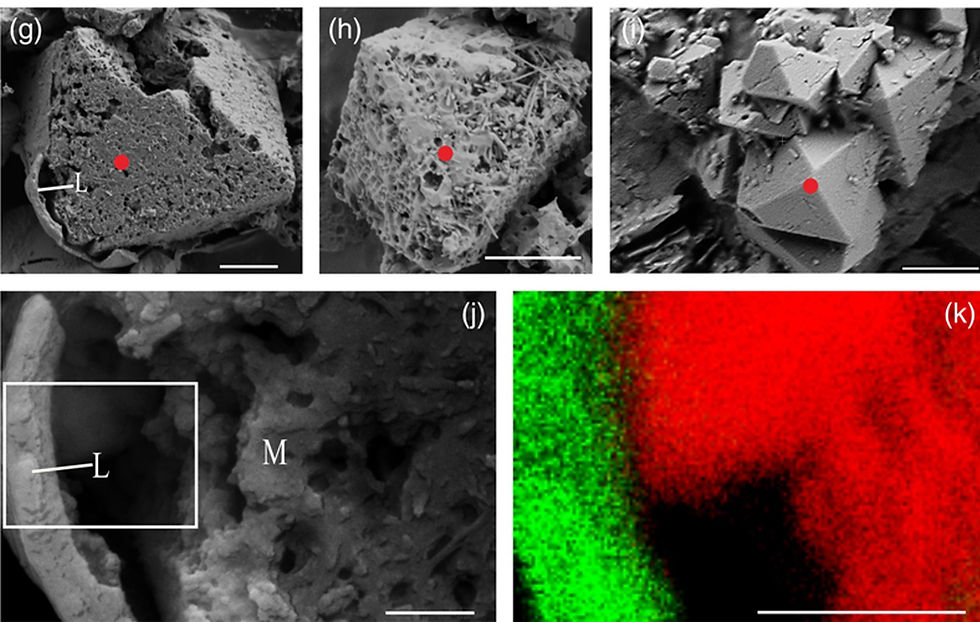
Figure 2: This shows the magnetite produced by R. bilocularis. The porous and octahedral structure of this magnetite can be seen (g, h, i, where scale bars are 2μm). The carbon-containing membrane (L) and the magnetite structures (M) are present in (j). (k) denotes elemental mapping of the white square shown in (j), where the red represents iron and green is carbon. Both (j) and (k) have scale bars of 0.5 μm.
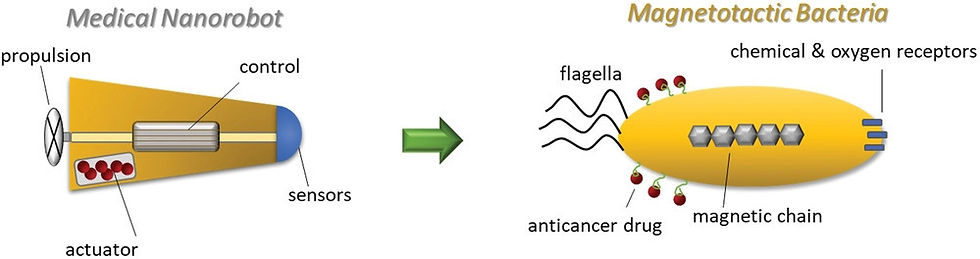
Figure 3: This shows the required features of a medical nanorobot compared to the biological, features of the magnetotactic bacteria. These MTBs can be paired with a targeted magnetic field for navigation. Note the MTBs ability to easily secure anticancer drugs and molecules to its surface.
A Working-Class Microbial Compass Needle
MTBs have proven effective in fulfilling a variety of nanobiotic roles in both artificial and microvasculature environments. Traits like their chemical purity, low toxicity, and surface morphology have caused some researchers to turn to bioengineering microbes for biomedicine and healthcare, rather than using artificial nanorobots to perform complex procedures. Magnetotactic microbes and their magnetosomes provide a means to control and direct the microbes to specific targeted tissues by manipulating local magnetic fields (14, Fig. 3). For example, it was found that an MTB, Magnetococcus marinus, could penetrate an anoxic tumour further than passive, artificial agents [15], in addition to bearing no negative effects. However, while microbial nanorobots such as MTBs are often more effective than their artificial counterparts, they are currently obstructed by their own physiology. For example, while the cell may often grow faster in relatively oxygenrich environments, magnetosome growth is optimised in oxygen-poor environments [16]. Meanwhile, magnetotactic eukaryotes have the potential to provide solutions for MTB shortfalls. The highly ciliated Tetrahymena pyriformis is a magnetotactic protozoan that reaches greater speeds than MTBs, and like MTBs, can be controlled via artificial magnetotaxis [17].
As well as the repurposing of the MTB as nanorobots, their magnetosomes can also be of use when extracted and replicated. The magnetosome can be bound to the drug and targeted to specific tissues, as shown by the coupling of antitumor drug doxorubicin to the isolated magnetosomes of Magnetospirillum gryphiswaldense [18]. Another study [19] also found that magnetosomes could be used for hyperthermia cancer treatments4 . Magnetic hyperthermia heats magnetic nanoparticles in a controlled manner to deactivate or kill cancer cells. This method efficiently destroys the tumour by utilising chains of magnetosomes, compared to the limited anti-tumour activity of individual magnetosomes. Alternatively, bacteria like Magnetospirillum gryphiswaldense can increase temperature to 45°C by applying an external magnetic field. M. gryphiswaldense has no direct impact on the viability or proliferation of the cancer cells, but rather its effect occurs only through its heating (i.e. the hyperthermia treatment) [14]. In addition to biomedicine, isolated magnetosomes can also be used to detect and separate pathogens in food (e.g. Salmonella [20]), and in key laboratory processes like protein assays, enzyme immobilisation, and as MRI (magnetic resonance imaging) contrast agents. Finally, MTB cultures (or isolated magnetosomes) can be used to generate a small amount of electricity through the application of Faraday’s law [21]. This study observed the movement of magnetic nanoparticles through a solenoid (a wire coil); while the electricity produced is minute, it may still have applications in bio and nanotechnology.
Magnetotactic microbes are typically motile, gramnegative bacteria that inhabit aquatic and endobenthic environments. Their use of magnetosomes to create an internal compass allows them to navigate to optimal oxygen conditions, providing an advantage over microbes that rely on Brownian movement. However, magnetotaxis is also present in protozoa; while this ability is typically the result of symbiotic relationships with the magnetotactic bacteria, some have developed the ability to independently biomineralise their own magnetosomes, thus redefining understanding around magnetotaxis, magnetosomes, and magnetotactic microbes. Both magnetotactic bacteria and eukaryotes ultimately have the potential to be beneficial in a range of biotechnological applications in the future.
¹ Brownian movement is the random movement of microbes which results in chance encounters on ideal environmental conditions.
² Microaerobic environments have very low concentrations of oxygen, while anaerobic environments are devoid of oxygen. This corresponds to different microbial respiratory environments.
3 These non-flagellated bacteria cannot be called magnetotactic bacteria because they cannot independently move in regard to the magnetic field lines, and instead must rely on the host eukaryotes.
4 Hyperthermia cancer treatment heats tissue to 45°C to impair or kill cancer cells, thus increasing the susceptibility of these cells to radio and chemo-therapy.
Comments