Explained: Dark Matter
- Caleb Todd
- May 4, 2021
- 9 min read
Updated: Feb 7, 2022
The concept of Dark matter explained by Caleb Todd
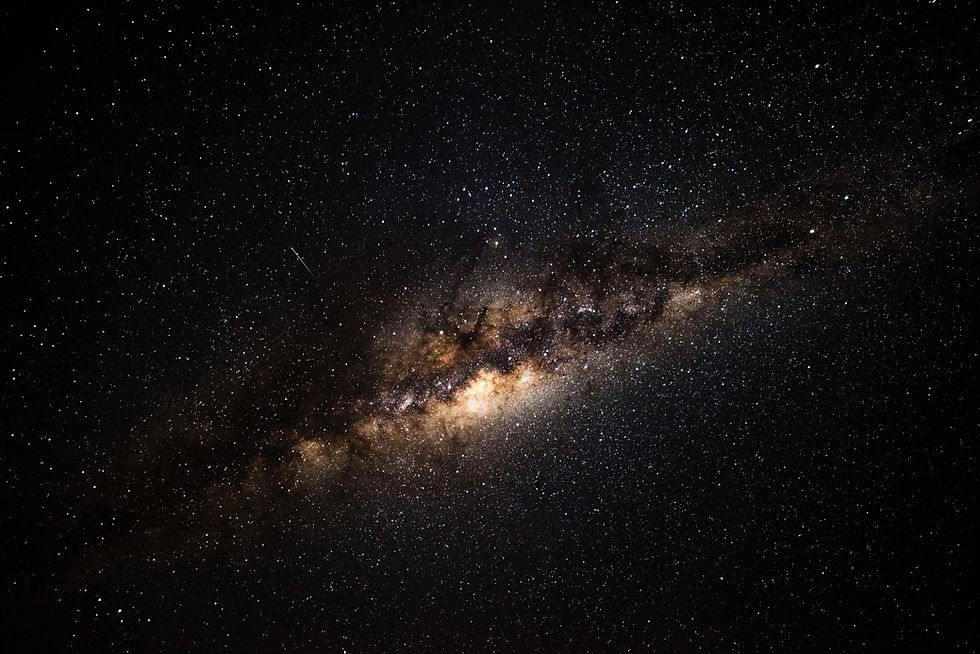
Photo by Brett Ritchie on Unsplash
Chances are, you’ve heard of dark matter. Whether in popular science articles or the technobabble of your favourite sci-fi show, it pops up pretty much everywhere, and no
wonder. Learning that what we think of as ‘normal’ matter only makes up 15% of the matter in the universe, and that the remaining 85% is comprised of some mysterious substance which we can’t touch or interact with in normal ways is quite the bombshell. It makes perfect sense that it would occupy so much of our science consciousness; it’s a concept at the very boundaries of what we know and understand, whose absurdity is easy to explain, and which has a catchy name and pub-quiz-style facts associated with it.
Perhaps the strangest part of dark matter, though, is that you’ve probably never been told where these crazy ideas come from. Dark matter is widely known but not widely understood. That may be why you’re reading this article. The natural assumption is that anything which can stump the best physicists of our generation must be so advanced that it will be beyond the reach of anyone without a PhD. In reality, though, dark matter can be understood by anyone who’s spun something in a circle and has experienced gravity. I’m guessing that’s most of us.
By the end of this article, you will understand why scientists treat an idea as ridiculous as dark matter seriously. To get there, though, we need to make sure you know enough about swinging things in circles and experiencing gravity.
Swinging Things in Circles
In 1686, Isaac Newton revolutionised physics when he laid out his three laws of motion (although we only need the first one). Newton realised that objects move in a constant direction at a constant speed unless there is a force acting on them. This can be unintuitive because you’ve never set something in motion that has kept going forever, but that’s only because forces like friction and air resistance slowly sap a moving object’s energy. If you threw a ball out in deep space, far from any gravitational pull or atmosphere, it would keep moving in the direction you threw it and at the same speed. That’s Newton’s first law in action. Conversely, if you threw the ball here on earth, its path would curve because gravity acts on it as a force. So the first law tells us that to curve an object’s path, we need a force acting on it.
But we can go further: it is possible to work out precisely what force is required. Suppose you were to tie a weight to the end of a string and try to spin it around. After a bit of experimentation, you would figure out that three factors influence how hard you have to hold onto the string to keep the mass from flying off in a straight line. These are: how heavy it is, how fast you’re swinging it, and how long the string is. Without going into the details, there is an exact relationship between the force on the object, the mass of the object, its speed, and the radius of the circle in which it moves. If you know the speed, mass, and radius involved in any circular motion you observe, then you know exactly what the force is without needing to measure it.
That’s everything you need to know about circular motion to understand dark matter. It’s a very straightforward idea: knowing the speed, mass, and radius of things going in a circle tells you the force acting on them. The other puzzle piece we need is gravity, which is the topic of the next section.
Gravity
As with circular motion (and, in fact, virtually all of physics), the story of gravity starts in earnest with Isaac Newton. He was the first person to formulate a universal theory of gravity, and it was a game-changer. Astronomy was arguably the biggest field of research at that time, and Newton showed that his theory of gravity could predict the planets’ behaviours perfectly.
A few generations before Newton, Johannes Kepler had observed that the planets all moved in ways that obeyed three laws of planetary motion. Kepler’s laws described the properties of celestial motion, but gave no justification for why they were so. They were observations lacking explanation. When Newton published his theory of gravity, he proved its validity by showing that Kepler’s three laws arise as natural consequences of the theory. Newtonian gravity solved arguably the most significant outstanding problem in physics at the time, so, naturally, the scientific community accepted it as correct. But the story doesn’t end there.
Over time, as we made more refined measurements and Newton’s theory of gravity was put to the test, people started noticing discrepancies. Newton’s predictions did not match perfectly with what we observed out in the universe. Most famously, in 1859, the precession of Mercury’s orbit was observed to be too large to be purely Newtonian. Ad hoc justifications were proposed, but they all ultimately failed to be satisfying solutions. On the one hand, Newton’s theory of gravity had been so successful in so many areas that it was difficult to discount it. On the other hand, it had serious gaps that couldn’t be ignored. A new theory was needed.
Enter Einstein.
In 1905, Einstein had his annus mirabilis, or ‘year of miracles’. Within the space of a few months, he published four papers that would revolutionise physics. One which decisively proved the existence of atoms, one on the quantum theory of light, one proposing the most famous equation ever (E = mc ), and one on his special theory of relativity, which redefined how we understood space and time. All in one year. It then took him ten more years to derive his general theory of relativity.
In essence, Einstein’s general theory of relativity was his theory of gravity. It describes the force of gravity as the experience of curvature in the spacetime continuum. When a moving object’s path is bent by gravity, it’s because it is moving in a straight line through curved space, not because it’s moving in a curved line through flat space. If that doesn’t make sense, then don’t worry because it really shouldn’t. The critical point is that the general theory of relativity is a theory of gravity, and — as you may have guessed — it does not suffer the shortcomings of Newtonian gravity. The precession of Mercury’s orbit aligns perfectly with general relativity’s (GR’s) predictions. Every test thrown at GR has validated its veracity. In fact, some of the most well-known and widely-discussed areas of modern physics are direct consequences of Einstein’s theory. The observation of gravitational waves, for instance, was among the final predictions of GR to be experimentally observed. Black holes, too, arise as solutions to Einstein’s equations. In short, it can’t be beaten.
Now you know much more than you need to know about gravity to grapple with dark matter. The gist is that we have a robust theory of gravity standing up to every prediction we can throw at it. Well, almost every prediction.
The Problem
Now we finally get to tie our two concepts together. We know that objects can move in circles given the proper force, and the size of that force is entirely determined by the speed and mass of the object and the circle’s radius. We also know that general relativity is a well-established theory of gravity that can predict the gravitational forces on objects accurately. You may see where this is going.
Galaxies are made up of hundreds of billions of stars orbiting around their mutual centre. They effectively undergo circular motion, with gravity acting as the force keeping them bound. Astrophysicists can measure stars’ masses, speeds, and distance from the centre of the galaxy. They can also derive the gravitational force acting on all these stars by working out the galaxy’s total mass and where that mass is distributed. So, we can calculate the force required to make the stars move in a circle, compare that to the force Einstein predicts the stars to be experiencing, and find that they are equal.
As it turns out, they aren’t equal.
In several different measurements over a number of years, physicists noticed that the stars orbiting in galaxies were moving too quickly. In other words, the gravitational force we know must exist significantly exceeds our expectations. Einstein’s gravitational theory failed to predict the galactic forces acting on stars correctly. There are only two possibilities: either general relativity is wrong, or galaxies contain a lot more mass than we can see.
Dark Matter
General relativity’s success makes it difficult to dismiss, which forces us to consider the second option. The proposal is this: there is a new type of matter that does not interact with light. Its only measured influence on ‘normal’ matter is through gravity. This would explain why galactic gravitational forces are greater than what we would expect; they are subsidised by unseen matter that is covertly increasing the mass in galaxies. Physicists are very original, so they named the substance which doesn’t interact with light ‘dark matter’.
The conceptual route we’ve taken here to motivate their existence is only one of many. Mass of cosmological structures can be measured in many different ways, and they all point to the presence of missing matter. For example, gravitational lensing is a process where large masses (like galaxies) with strong gravitational fields bend light around them. We regularly observe gravitational lensing around clusters of galaxies, and the geometry of the lensing allows us to estimate the mass involved. Again, we find that there is more mass in galaxies than we can see. A host of different observations all support this same thesis.
However, that is not the end of the story. As elusive as dark matter may be, it does have (feeble) effects on other matter. We should, in principle, be able to detect it directly. Indeed, many researchers are trying to do precisely that. As of yet, though, none have been successful. On the one hand, this is not unexpected. Any direct interaction between a dark matter particle and normal matter will be so slight that incredible experimental precision is needed. Furthermore, there is considerable ambiguity over exactly what properties dark matter would have and where we should look, meaning detection efforts have to be aimed at a broad range of candidate properties. Nonetheless, a lack of direct evidence for these particles is a valid objection to their veracity. That takes us back to the other possibility: general relativity could be wrong.
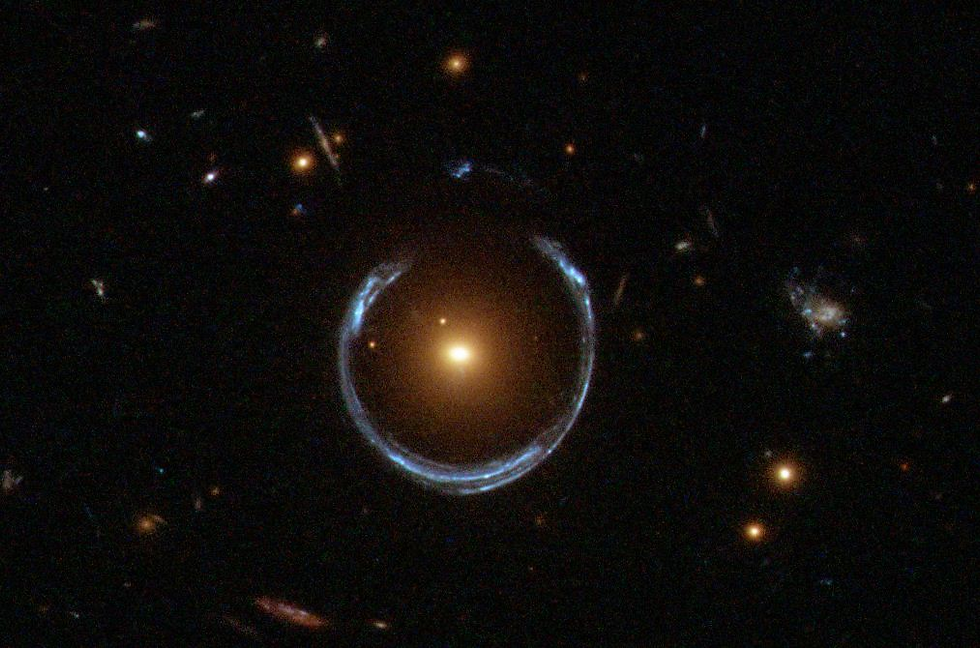
An observation of gravitational lensing by the Hubble space telescope. The orange light in the centre is a large galaxy. Behind it is a blue galaxy whose light is bent around the red galaxy in all directions because of gravity, causing the illusory ring effect. The precise position and shape which the lensed image takes on depends on the gravitational field strength, which, in turn, depends on the mass of the red galaxy and where the mass is distributed. Such observations have provided evidence for the existence of dark matter.
A New Theory of Gravity
Let’s come back to Newtonian gravity. We said that it was a revolutionary theory that was taken on board because of its startling ability to predict otherwise unexplained phenomena. But, over time, discrepancies arose between observations and expectations that forced us to look elsewhere for our theory of gravity. Now let us consider general relativity. GR was a revolutionary theory that was taken on board because of its startling ability to predict otherwise unexplained phenomena. But, over time, discrepancies have arisen between observations and expectations. Sound familiar?
Admittedly, dark matter is a more satisfying solution to general relativity’s problems than anything proposed for Newtonian gravity’s. However, there is a second strike against GR’s name which redoubles the doubts over it: we already know it’s wrong. This may seem surprising after I spent so much time talking about how it has passed every test thrown at it, but at the time, I was omitting an important detail: general relativity has only really been tested on particular scales. We know it works incredibly well for objects the size of planets or stars, but it may no longer apply at larger scales. Newtonian gravity worked incredibly well for objects the size of you and me, but it began to fail when considering things the size of our solar system. Testing a theory in one regime offers no guarantees of its validity in another regime.
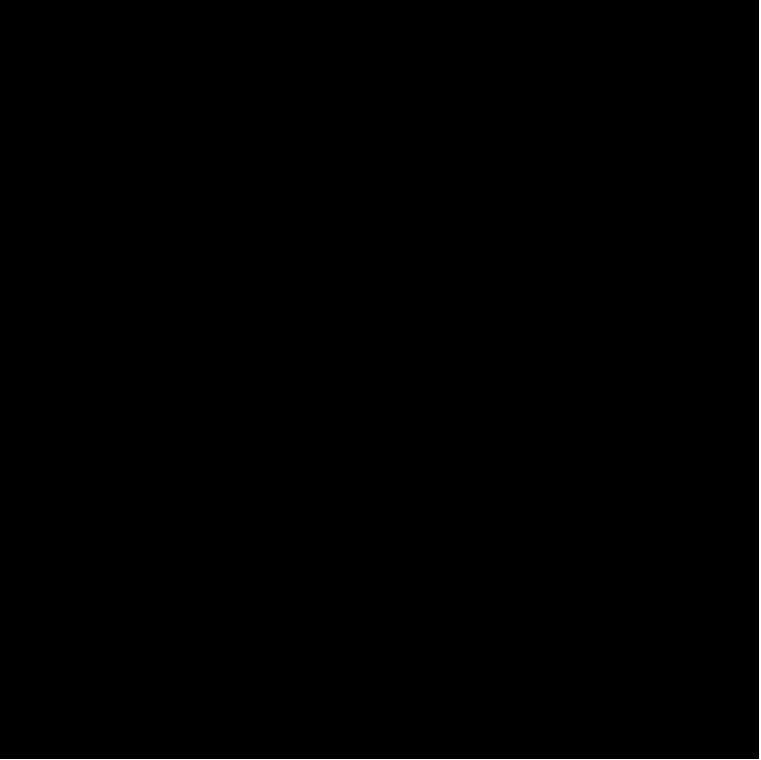
A computer-generated image which approximates what some scientists believe dark matter would look like. This is only a theoretical conjecture, though, as we are yet to directly detect any dark matter.
The problems for general relativity get worse, though. GR is one of two theories central to modern physics; it describes things on large scales, while quantum field theory describes things on small scales. Both theories have been highly robust within their own domains, but they cannot be reconciled with each other. There is no definitive quantum theory of gravity that would unify physics under a single conceptual framework. General relativity simply cannot be reconciled with quantum field theory at small scales. Quantum field theory is the best supported theory in science - its predictions have been proven to a level of accuracy beyond any others’ - so we know that GR cannot be entirely correct.
Having said that, GR being an imperfect theory does not imply that dark matter does not exist. To dismiss dark matter, we would first have to find a theory of gravity that explains the phenomena that made us consider dark matter in the first place. Many attempts have been made at correcting GR. Some of these attempts have successfully explained some of the phenomena, but none have explained all of them. The single proposal of dark matter can explain a huge variety of observations, and Occam’s razor suggests that a single, simple proposition that is consistent with many observations is more likely to be correct than a large number of complex solutions which partially explain some observations.
For this reason, dark matter is the more widely accepted solution to the problems which astrophysicists face. Still, until it is directly observed, the question mark over dark matter remains, and physicists will continue to debate its veracity. You can judge which side of the debate you sit on.
Comments