Janus Nanoparticles and Self Assembly
- Caleb Todd
- Sep 27, 2021
- 6 min read
Updated: Feb 7, 2022
Caleb Todd
Scientific and technological progress is not unrestrained. There are bottlenecks that slow or even prevent progress in certain fields of research, like funding or the number of researchers working in the field. One constraint which I think we are less aware of is the materials we have available. Edna Mode in The Incredibles makes super suits that allow the protagonists to utilise their powers without inhibition. Elastigirl’s can stretch, Violet’s turns invisible, and so on. In the same way, real-world scientists couldn’t realise their ideas without suitable materials to work with.
An example close to all of our hearts is the internet, which uses laser light travelling through fibre optic cables to transmit information. Fibre-optic cables are glass, but you can’t just heat up sand and expect to get something usable. Impurities have to be removed, new impurities have to be added (called dopants), and stretching and layering to give cables the desired internal structure are all essential to keeping the light going where you want it to with as little loss as possible [1]. Lasers are even more remarkable. Though their principle of operation was theorised by Einstein in 1916, the first laser was only operational in 1960 [2]. Getting a laser with a specific colour requires tuning properties at an atomic level. The internet is completely dependent on the production of materials with the exact properties we need, and it is not the only such example.
Some materials we have developed seem nearly miraculous in their utility, and more are being invented all the time. Living at the intersection between physics, chemistry, and engineering, materials scientists generate these new and interesting materials and study their properties. However, some material properties simply cannot be achieved with our current understanding. For example, one of the biggest roadblocks on our route to quantum computing is the absence of feasible room temperature superconductors. Superconductors are materials that can carry electricity without energy loss [3], and they are central to many quantum computer architectures [4]. However, materials generally only exhibit superconductivity at very low temperatures which are expensive to create and maintain. Those that don’t will require incredibly large pressures that are equally impractical. There are many other examples of the material properties we can’t access, limiting our technology, which is why materials science is so pivotal to scientific advancement.
An exciting paradigm in material development is so-called nanoparticle self-assembly. A nanoparticle is an object on the scale of one billionth of a metre — made of only tens of atoms. If you construct nanoparticles with the right properties and under the right conditions, their interactions with each other can cause them to spontaneously order themselves [5]. Two might join to become a pair, then the pairs will join together, and so on, forming larger and larger structures. A ubiquitous example would be lipid molecules with one hydrophobic (water-repelling) end and one hydrophilic (water-attracting) end. When many lipids are immersed in water, they will clump together to hide the hydrophobic ends from the surrounding fluid [6]. The exact configuration depends sensitively on the specifics of the inter-particle interactions and the conditions to which they are exposed.
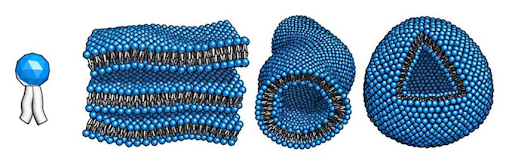
Figure 1: Different patterns of formation into which lipids can spontaneously develop. Image taken from [6].
If the nanoparticles are designed well the self-assembly process can result in macroscopic materials. An attractive part of this approach to materials science is sustainability. Since the materials are built up through a spontaneous process, they are easily recyclable. Should one product come to the end of its use, its material can be broken down and sent through the self-assembly process again to build an entirely new product. Even better, the properties of these materials (like stiffness or density) can be customised by tweaking the nanoscale structures. In this way, nanoparticle self-assembly offers an exciting route towards developing materials with new and desirable properties, unlocking new regimes for technology and scientific experimentation.
The question, then, is this: how do we predict the macroscopic properties of a self-assembled material, and how do we align them with our desires? The answer, usually, is to return to those nanoscopic interactions that gave rise to the self-assembly in the first place. We study the bonds which form between two nanoparticles and build up from there.
Last year, I took part in a research project which analysed Janus nanoparticles in exactly that manner. Janus is the Greco-Roman god with two faces, so Janus nanoparticles are nano-scale objects with two distinct sides. For example, you might have one side positively charged and the other negatively charged. I was studying an amphipathic Janus nanosphere: a spherical nanoparticle that is half hydrophilic and half hydrophobic. Just like lipids, if there is a pair of amphipathic Janus nanospheres in a fluid, their hydrophobic parts will make contact to hide each other from the surrounding water (as shown in Figure 2). Such a pairing is called a Janus dimer, and it forms the first building blocks in a self-assembly process [7].

Figure 2: Molecular dynamics simulations of two Janus spheres immersed in a fluid. The blue atoms are hydrophobic, red are hydrophilic, and turquoise are the fluid. Image taken from [7].
In my project, I analysed these Janus dimers, studying the orientations in which they preferentially configure. We might expect the dimer to most frequently sit end-on-end. This would seem, in some sense, to hide the hydrophobic surfaces the most. Another valid guess may be for any orientation to be equally probable, as long as the contact point was between hydrophobic sides. As we know, however, reality is often disappointing; the answer is more complex.
There are two competing effects dictating what configurations Janus dimers will prefer to reside in: energy and entropy. Energy is linked to my colloquial description of hydrophobic surfaces ‘wanting’ to hide from the water. In more rigorous terms, configurations where the hydrophobic sides are exposed to fluid have higher energies, which is less favourable. Entropy can be thought of as the number of ways a given configuration can form. To illustrate this, consider two coin flips. The possible outcomes are HH, HT, TH, and TT, and all are equally probable. However, a configuration with one head and one tail can be reached in two different ways, HT and TH, and is consequently more probable than the configuration with two heads, or that with two tails. Janus nanoparticles (in fact, all physical systems) exhibit similar properties. Some configurations can be formed in more than one way — so-called ‘degenerate’ states — and are consequently more probable.
The energetic factor alone would, in fact, prefer the end-on-end configuration. That is the state where the hydrophobic surfaces are furthest from the fluid on average, which is energetically desirable. However, entropy ruins this heuristic. Contact points nearer the equator of the Janus sphere (where the hydrophobic and hydrophilic hemispheres meet) have a larger degeneracy because there is more available surface area than at the poles. Indeed, there is only one point at which the spheres could make contact at the poles: when they are perfectly end-on-end; but if the spheres meet at the equators, they have an entire circle of points available to them. So, energy makes the Janus spheres want to make contact end-on-end, and entropy wants them to meet at 90 degrees away at their equators. As you can imagine, these two competing influences balance out somewhere in the middle. Figure 3 shows that the preferred state is about 45 degrees turned out from the poles.
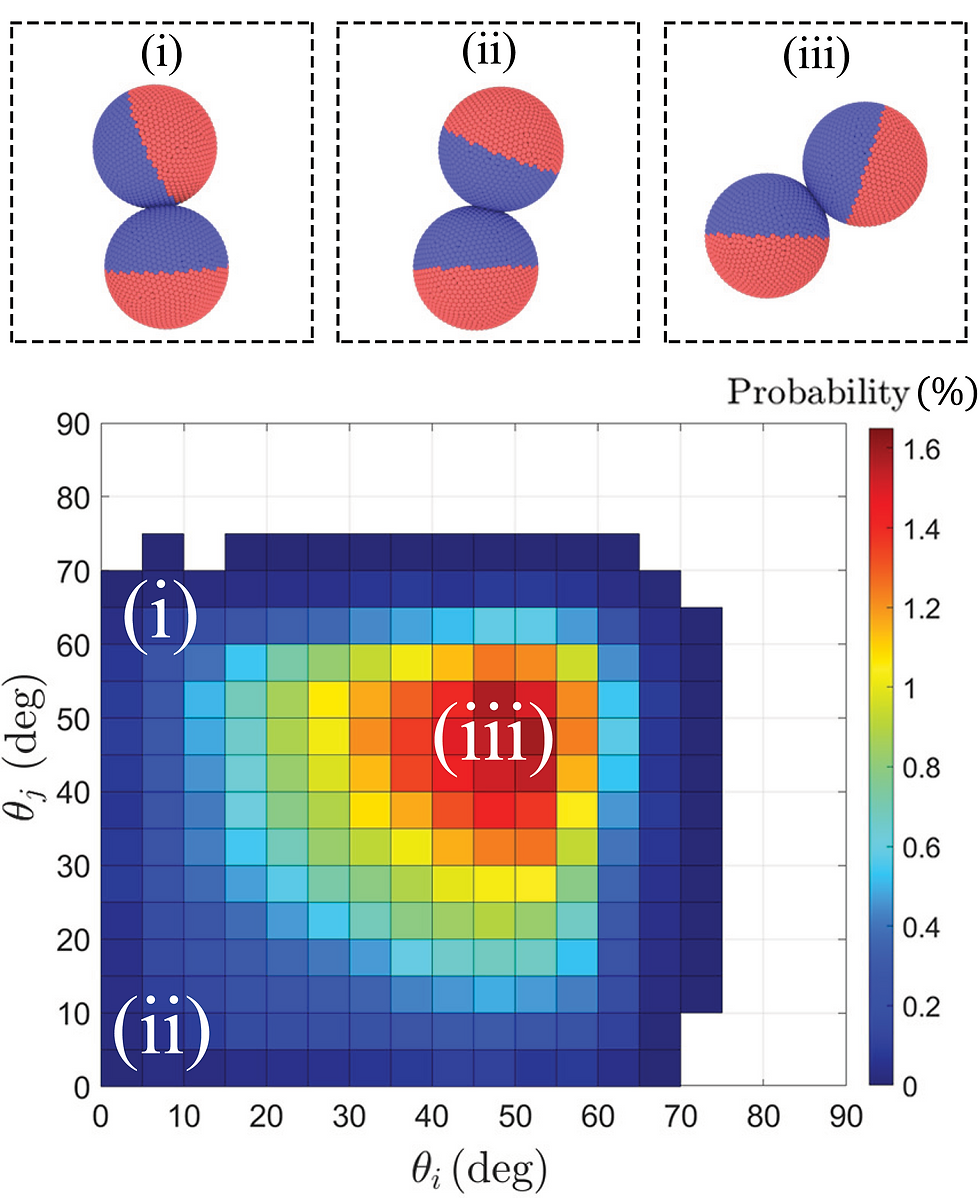
Figure 3: The configuration probabilities as the two spheres (i and j) are rotated out from their connecting axis. The bottom left corner (ii) is perfectly end-on, and the top right corner would be side-on. The most probable configuration is at (iii), in-between these two extremes. Image taken from [7].
But let’s move back to the big picture. Once you understand these dimer interactions in a deep and fundamental way, you can scale them up. Thousands or millions of Janus particles’ interactions can be built as the aggregate of all pairwise interactions. Using this information, we can run simulations that predict what properties self-assembled Janus materials might have, and how those properties can be controlled. And that isn’t unique to Janus spheres; the same process can be replicated for other types of nanoparticles. With new materials comes new experimental capacity, new technologies, and a more sustainable future. Whether Janus spheres will unlock new regimes in physics or biology is yet to be seen, but the underlying principles certainly have that potential.
References
[1] T. Li, Optical fiber communications: fiber fabrication. Elsevier, 2012.
[2] A. J. Gross and T. R. Herrmann, “History of lasers,” World journal of urology, vol. 25, no. 3, pp. 217–220, 2007.
[3] M. Cyrot, “Ginzburg-landau theory for superconductors,” Reports on Progress in Physics, vol. 36, no. 2, p. 103, 1973.
[4] H.-L. Huang, D. Wu, D. Fan, and X. Zhu, “Superconducting quantum computing: a review,” Science China Information Sciences, vol. 63, no. 8, pp. 1–32, 2020.
[5] M. Grzelczak, J. Vermant, E. M. Furst, and L. M. Liz-Marźan, “Directed self-assembly of nanoparticles,” ACS nano, vol. 4, no. 7, pp. 3591–3605,2010.
[6] S. Yang, Y. Yan, J. Huang, A. V. Petukhov, L. M. Kroon-Batenburg,M. Drechsler, C. Zhou, M. Tu, S. Granick, and L. Jiang, “Giant capsids from lattice self-assembly of cyclodextrin complexes,” Nature communications, vol. 8, no. 1, pp. 1–7, 2017.
[7] S. Safaei, C. Todd, J. Yarndley, S. Hendy, and G. R. Willmott, “Asymmetric assembly of Lennard-Jones Janus dimers,” Phys. Rev. E, vol. 104, p. 024602,Aug 2021.
Commentaires